1. Introduction
Postnatal mammalian skeletal muscle growth, maintenance and regeneration after damage is mostly dependent on the activity of satellite cells (SCs), the resident stem cell population [
1,
2]. In healthy muscle, these mononucleated cells are located in a specialized niche at the periphery of adult striated multinucleated myofibers (hence the name) between the sarcolemma and the basal lamina ensheathing each myofiber, in a dormant state. In response to different biochemical and mechanical stimuli emanated by the surrounding growing or injured microenvironment, SCs become activated, exit the quiescent state and start to proliferate and differentiate essentially recapitulating embryonal myogenesis steps [
3,
4,
5]. SCs are characterized by the expression of the paired box transcription factor Pax7, regarded as the canonical SC-specific marker required for their survival and functionality and by the expression of the myogenic regulatory factors (MRFs) Myf5, MyoD, MRF4 and myogenin. In particular, when quiescent, SCs express Pax7 and the myogenic transcription factor Myf5 (detectable in ~90% of quiescent SCs). Once activated, they undergo mitotic division to give rise to a progeny of proliferating adult myoblasts co-expressing Pax7, Myf5 and the myogenic determinant factor 1 (MyoD), the master regulator of SC proliferation/activation. Afterwards, most of these cells lose Pax7, maintain MyoD expression (Pax7
+/MyoD
+) and progress into the myogenic program acquiring myogenin and MRF4/Myf6 expression to differentiate into skeletal myocytes. These, in turn, fuse to one another forming myotubes and finally new myofibers. At the same time a small population maintains Pax7 and down-regulates MyoD (Pax7+/MyoD−) and self-renew, not executing the myogenic program and reconstituting a compartment of quiescent ‘stem cell’ able to respond to future demand [
6]
. It has been demonstrated that SCs, as other stem cells, undergo an energy metabolic reprogramming during differentiation, consisting of a shift in metabolic substrate utilization. In particular, it has been shown that proliferating myoblasts mainly depend on glycolytic energy production differently from myotubes that exhibit an increased aerobic capacity [
7,
8,
9]
. Recent studies have highlighted the Hypoxia-inducible factor (HIF)-1α as a regulator of myogenesis and SCs/myoblasts’ homeostasis in vitro and in vivo [
10,
11,
12,
13,
14,
15,
16,
17,
18,
19,
20,
21,
22]
. It is a dimeric basic helix-loop-helix-PER-ARNT-SIM transcriptional factor consisting of a constitutively expressed subunit HIF-1β and an oxygen-regulated/sensitive subunit HIF-1α (or its paralogs HIF-2α and HIF-3α). HIF-1α biological activity depends on post-transcriptional modifications that are responsible for the α-subunit stability and activity. Usually in normoxia, post translational modifications of HIF-1α (HIF-1α is hydroxylated by the active prolyl-hydroxylases -PHDs-) lead to the subunit degradation via ubiquitin-proteasome pathway. By contrast, in hypoxia PHDs are inhibited, HIF-1α cannot be hydroxylated and becomes stable. It translocates to the cell nucleus, where it forms a transcriptional complex with HIF-1β which is able to interact with different coactivators and to regulate the expression of several target genes involved in many cellular processes including regulation of glycolytic metabolism in skeletal muscle [
15,
23,
24,
25]. However, in skeletal muscle tissue and SCs an oxygen level-independent regulation of the expression and activation of HIF-1α has been observed thus suggesting a role of such a protein in skeletal muscle physiological functions and its homeostasis. Nevertheless some controversy remains on the spatio-temporal expression of HIF-1α and on its exact role in myoblasts during myogenic differentiation. In addition, the knowledge of molecular targets of this factor needs to be expanded [
10,
17,
18,
19,
21,
22,
26,
27,
28,
29,
30,
31,
32,
33].
The present study was undertaken to better clarify the role of HIF-1α in myoblasts undergoing myogenic differentiation under normoxia conditions and to evaluate MMP-9 as a downstream effector of HIF-1α. In particular, we investigated MMP-9 as target gene when considering that MMP-9 has been demonstrated to be a target of HIF-1α in different cell types [
34,
35,
36,
37] and the role of MMP-9 in skeletal myogenesis has been documented [
38,
39,
40,
41].
This research demonstrates that: i) the expression of HIF-1α synchronized with that of MMP-9 peaking at early phase of differentiation (24 h) concomitantly to that of MyoD ii) MMP-9 was a target of HIF1-α and iii) HIF-1α/MMP-9 axis was necessary for the morpho-functional myogenic activation/commitment of myoblasts.
4. Discussion
The current study provides experimental evidence that HIF-1α plays a critical function during myoblast differentiation in vitro that is not strictly related to hypoxic conditions. In fact, we have uncovered a previously unrecognized relationship between HIF-1α and MMP-9 in myoblasts induced to differentiate under normoxia conditions. In particular, we have evinced the essential role of such an axis in the very early phase of the normoxic myogenic progression, namely activation and commitment. These conclusions arise from the following observations.
First, morphological and biochemical analyses showed a different spatial and temporal expression of HIF-1α protein in the C2C12 cells undergoing differentiation: in particular HIF-1α expression increased after 24 h of culture in differentiating condition (DM) as compared to those observed in the cells in growing condition (PM) and declined with the differentiating time. Interestingly, HIF-1α expression is synchronized with that of myogenic activation marker MyoD and with Notch-1, a key determinant of myoblast activation and proliferation. Proliferating committed mononuclear myoblasts exhibited a more marked nuclear expression of HIF-1α as compared to the more elongated cells and polynucleated myotubes. The expression pattern of HIF-1α was confirmed on murine primary SCs. Conversely Wagatsuma et al. 2011 [
30] found that C2C12 cells showed a decrease of HIF-1α protein expression when switching from growth to differentiation medium, whereas Ono et al. 2006 [
21] showed that the HIF-1α protein was hardly detected in undifferentiated C2C12 myoblasts cultured in growth medium. This discrepancy may be attributed to different cell culture experimental conditions. However, according to our findings and other previous research [
19,
29], these papers [
21,
30] demonstrated the nuclear expression of HIF-1α in the early stage (induction) of C2C12 cell myogenesis process. In myoblasts the nuclear HIF-1α expression may be consistent with the functional induction of the genes encoding glycolytic enzymes required to satisfy the cell energy demand in the early phase of myogenic differentiation process [
7,
8,
9,
19,
50,
51,
52]. Along this line, we have confirmed in our experimental myogenesis model that the cells undergo metabolic reprogramming during the differentiation process: in fact, proliferating myoblasts mainly depended on glycolytic energy production whereas myotubes showed a more developed aerobic capacity, as judged by the analyses of the expression of LDH-A and SDH-B. Moreover, during differentiation time we have observed an increase of the expression of PGC-1α that has been demonstrated to regulate LDH by decreasing LDH-A mRNA transcription and the enzymatic activity of pyruvate to lactate conversion [
53]. In addition, PGC-1α has been recognized as a crucial regulator of mitochondrial biogenesis. Indeed, a clear consensus is evident in the literature on the occurrence of mitochondrial biogenesis during myogenic differentiation so as to sustain the energy demand required for myotube formation and successively polynucleated long myofibers [
54]. Moreover, taking in mind the role of vascular endothelial growth factor (VEGF)/VEGF receptor-mediated signaling in myogenic differentiation [
47,
55,
56,
57] and the well-known cross-talk between HIF-1α and such signaling [
19,
58,
59,
60], we may also postulate the possibility that HIF-1α is physiologically involved in myoblast activation and proliferation by modulating VEGF signaling. Different from the protein expression, we have found that HIF-1α mRNA was constantly expressed in C2C12 myoblasts both under growth and differentiating steps according to previous data [
19,
21,
29,
30]. Our results indicate that the differences in protein level are not due to a different mRNA expression and suggest instead that the regulation of HIF-1α expression and activity during myogenic differentiation in vitro occurred at post-transcriptional level, possibly including protein transduction and stabilization/degradation, nuclear localization and transactivation.
The hypoxia-independent expression of HIF-1α in myoblastic cells during differentiation is not surprising as demonstrated in previous research [
21,
22,
30]. This observation is also in line with the increasing evidence showing that HIF-1α is involved in different biological functions requiring its activation under normoxic conditions. The exact reason why HIF-1α escapes proteasome degradation in our cell model is not explained. However, non-hypoxic activators of HIF-1α have been described including, among others, nitric oxide NO [
61,
62] demonstrated to be produced by C2C12 myoblasts, peaking within 24 h after the induction of differentiation [
63]. Moreover, the stability of HIF-1α in skeletal muscle cells depends on different regulators including not only the oxygen sensors named prolyl hydroxylases PHDs that guide the oxygen-dependent degradation of HIF-1α, but also the heat shock protein 90 (Hsp90) whose content and expression is independent on oxygen concentration. In particular, it has been observed that Hsp90 protects HIF-1α from proteasome degradation promoting its accumulation during in vitro differentiation of myoblastic cells under normoxia [
21,
22]. A PCG-1α dependent stabilization of HIF-1α has been also proposed in skeletal muscle cells [
64].
Furthermore, the observed cytoplasmic expression of HIF-1α, may suggest an unconventional function of HIF-1α, as a signaling regulator distinct from its canonical activity as a transcriptional factor [
65,
66,
67]. The specific mechanisms modulating HIF-1α expression needs further exploration.
The potential critical role of HIF-1α during the early phase of myogenesis under normoxia, suggested by our first results, has been then confirmed by experiments in which HIF-1α gene expression was silenced by specific siRNA. Indeed, silenced cells cultured in myogenic differentiation medium showed a reduction of the activation markers tested, namely Ki67, Notch-1 and MyoD. Taking in mind that during the process of myoblast differentiation, MyoD expression increases at first to decrease then [
68], as confirmed in our experimental model, our results might also suggest a more differentiated state of the silenced cells and, in turn, the possibility that HIF-1α could function counteracting differentiation. However, morphological analysis uncovered that silenced cells did not show morphological features of more differentiated cells such as elongation, alignment or fusion into myotubes, leading us to conclude that HIF-1α is just required for cell activation/commitment. Our findings are consistent with previous data demonstrating that siRNA-mediated knockdown of HIF-1α in C2C12 myoblasts results in inhibiting myotube formation [
21] and with data from Cirilli et al [
15] showing that the pharmacological activation of HIF-1α by the treatment with some PHDs inhibitors promoted C2C12 myoblast differentiation. In contrast, it has been reported that the constitutive expression of active HIF-1α in C2C12 myoblasts did not accelerate differentiation such as the ectopic expression of DEC1/Stra13, a target gene of HIF-1α, did not prevent C2C12 myotube formation under either hypoxia or normoxia [
69], supporting the notion that HIF-1α is not directly involved in postnatal myogenesis. Nevertheless, all these observations may suggest the requirement of a coordinated temporal modulation of HIF-1α and thus it cannot be excluded that:
i) HIF-1α functionality may be critical just for some steps of the myogenic process,
ii) HIF-1 may act differently during the differentiation period. Therefore, the above cited papers are not in contrast with the findings of the present study. However the role of HIF-1α in the late phase of myogenesis in our model remains to be investigated.
A novel finding from this study is the demonstration that MMP-9 is a downstream target of HIF-1α and that HIF-1α/MMP-9 signaling pathway is involved in myoblast activation. Indeed we found that MMP-9 expression synchronized with that of HIF-1α showing higher level after 24 h of differentiation, and that myoblasts silenced for HIF-1α exhibited a reduction of MMP-9 expression. Of note, the gene silencing of MMP-9 or its pharmacological inhibition caused a decrease of the activation markers of myogenesis similarly to HIF-1α silencing, thus suggesting an unconventional intracellular action of such proteases. In this line, a previous study conducted by our research group unraveled that bone marrow mesenchymal stromal cell secretome was able to increase MMP-9 expression in murine myoblasts and that this event was accompanied by an increase of cell mobilization, proliferation and activation [
40]. Consistent with our findings, an earlier study demonstrated that inhibition of MMP-9 (with doxycycline and anti-MMP-9) delayed in vitro proliferation and differentiation of satellite cell- derived myoblasts from rat Soleus muscle [
70]. The same research group documented that rat proliferating myoblasts exhibited a nuclear expression of MMP-9 and that MMP-9 activity was required for proper cell cycle progression, since its inhibition affected myoblast proliferation [
38,
41]. Different from Zimowska et al. we did not observe a nuclear localization of the protein suggesting that in our experimental model MMP-9 is not directly involved in nuclear events. Based on the observed distribution of MMP-9 that appeared linearly organized along filamentous structures, most likely cytoskeletal ones, we may hypothesize the occurrence of a MMP-9-dependent cytoskeletal rearrangement required for driving myoblast activation and differentiation [
49,
71,
72].
A strength point of this research is that the outcomes from biomolecular, biochemical and morphological analyses, were robustly supported by the functional electrophysiological recordings. In particular, the analysis of the passive properties of the committed myoblasts focused on those parameters supposed to be indicative of a more differentiated phenotype. Accordingly, the cell capacitance (Cm), which is an index of the cell surface, is expected to increase during differentiation [
73,
74]. This was actually observed in our cells induced to differentiate, but when HIF-1α expression was silenced or MMP-9 was inhibited, its values turned out to be similar (not statistically different) to those measured in proliferating (not differentiating) myoblasts. Analogous observations could be made for the analysis of the specific conductance (Gm/Cm), that can be considered another index of differentiation [
75]. Also preliminary results on resting membrane potential, that is supposed to become more polarized in myotubes than in myoblasts, confirmed these indications (data not shown). Finally, we intended to estimate the ion current flowing through voltage-activated Ca
2+ channels. This, in fact, can be considered as a typical feature of skeletal muscle differentiation for its crucial role in excitation-contraction (EC) coupling in adult skeletal muscle fibers [
76]. Although the structure of skeletal muscle L-type calcium channels consists of the pore forming α1 subunit, and the α2δ, β, and γ subunits, interestingly, these subunits are usually not expressed simultaneously during differentiation of myotubes in vitro [
77,
78,
79]. This fact may undoubtedly hamper the early acquisition of fully functional voltage-dependent properties and their consequent clear revelation by electrophysiological techniques. Indeed, in our study we could observe that untreated myoblasts (WT) grown in PM usually showed an inward not-inactivating current suggesting a scarcely voltage-dependent ion entry. In contrast, although 24 h might be a very early differentiation stage, myoblasts cultured in DM exhibited an inward current with an increased amplitude and a sort of inactivation at higher voltage steps, resembling the I
Ca time course of a more differentiated skeletal muscle cell. Again, current recorded from cells silenced for HIF-1α was very similar to that evoked in WT myoblasts in PM, suggesting that, although in DM, HIF-1α-silenced cells maintain the same ion channel profile/features shown by WT in PM. A similar result was observed when MMP-9 was inhibited by SB-3CT. This may suggest that HIF-1α and MMP-9 have a role in C2C12 myoblasts driving Ca2+/ion channels expression/assembly during the early differentiation phase.
This hypothetical role of HIF-1α may be supported by previously published data even if achieved in a different muscle tissue, namely in pulmonary arterial smooth muscle cells [
80]. The Authors reported that HIF-1α plays a key role in Ca
2+ homeostasis, especially/specifically/just modulating the expression of transient receptor potential channels (TRPCs), thus contributing to pulmonary vascular remodeling.
Author Contributions
“Conceptualization, F.C., A.T., R.S. and C.S.; methodology, F.C., A.T., R.S. and C.S.; validation, F.C., A.T., M.P., F.P., R.G., R.S. and C.S.; formal analysis, F.C., F.P. and R.S.; investigation, F.C., A.T. M.P., F.P., R.G., R.S., C.S.; resources, F.C., S.Z.-O., R.S, C.S.; data curation, F.C., A.T., F.P., R.S., C.S.; writing—original draft preparation, F.C., A.T., R.S., C.S.; writing—review and editing, F.C., A.T., M.P., F.P., R.G., S.Z.-O., R.S. and C.S.; visualization, F.C., A.T., R.S., C.S.; supervision, C.S.; project administration, R.S., C.S.; funding acquisition, F.C., S.Z.-O., R.S. and C.S. All authors have read and agreed to the published version of the manuscript.”
Figure 1.
Morphological analysis of C2C12 myoblast differentiation under normoxia. C2C12 cells were cultured in the proliferation medium (PM) for 24h or in myogenic differentiation medium (DM) for different times (24, 48, 72h and 5d). (A-L) Representative confocal immunofluorescence images of C2C12 cells immunostained with antibodies against (A-D) the nuclear protein Ki67 (green), (E-G) MyoD (green), (H) Myogenin (red) and (I-L) Notch-1 (green). (M,N) Quantitative analyses of Ki67 and MyoD positive nuclei expressed as the percentage of the total nuclei, respectively. (O) Densitometric analysis of Notch-1 fluorescence signal intensity (F.I.) in the indicated experimental condition, performed on digitized images in 10 regions of interest (ROI) of 100 μm2 for each confocal stack (10). a.u.: arbitrary units. (P) Representative phase contrast light microscopic image of myotube formation after 5d culture in DM. (Q,R) Representative confocal immunofluorescence images of C2C12 cells grown in DM for 5d immunostained with antibodies against myogenin (red) and sarcomeric actin (green) respectively. In (Q) the cells were counterstained with-phalloidin to visualize F-actin filaments (green). Scale bar: in (A-L), (Q) and (R), 25 µm; in (P), 200 µm. Data are reported as mean ± SD of at least three independent experiments performed in triplicates. Significance of difference: * p<0.05, ** p<0.01 vs PM; °° p<0.01 vs DM 24h; # p< 0.05, ## p<0.01 vs DM 48h (One-way ANOVA with post-hoc Tukey HSD).
Figure 1.
Morphological analysis of C2C12 myoblast differentiation under normoxia. C2C12 cells were cultured in the proliferation medium (PM) for 24h or in myogenic differentiation medium (DM) for different times (24, 48, 72h and 5d). (A-L) Representative confocal immunofluorescence images of C2C12 cells immunostained with antibodies against (A-D) the nuclear protein Ki67 (green), (E-G) MyoD (green), (H) Myogenin (red) and (I-L) Notch-1 (green). (M,N) Quantitative analyses of Ki67 and MyoD positive nuclei expressed as the percentage of the total nuclei, respectively. (O) Densitometric analysis of Notch-1 fluorescence signal intensity (F.I.) in the indicated experimental condition, performed on digitized images in 10 regions of interest (ROI) of 100 μm2 for each confocal stack (10). a.u.: arbitrary units. (P) Representative phase contrast light microscopic image of myotube formation after 5d culture in DM. (Q,R) Representative confocal immunofluorescence images of C2C12 cells grown in DM for 5d immunostained with antibodies against myogenin (red) and sarcomeric actin (green) respectively. In (Q) the cells were counterstained with-phalloidin to visualize F-actin filaments (green). Scale bar: in (A-L), (Q) and (R), 25 µm; in (P), 200 µm. Data are reported as mean ± SD of at least three independent experiments performed in triplicates. Significance of difference: * p<0.05, ** p<0.01 vs PM; °° p<0.01 vs DM 24h; # p< 0.05, ## p<0.01 vs DM 48h (One-way ANOVA with post-hoc Tukey HSD).
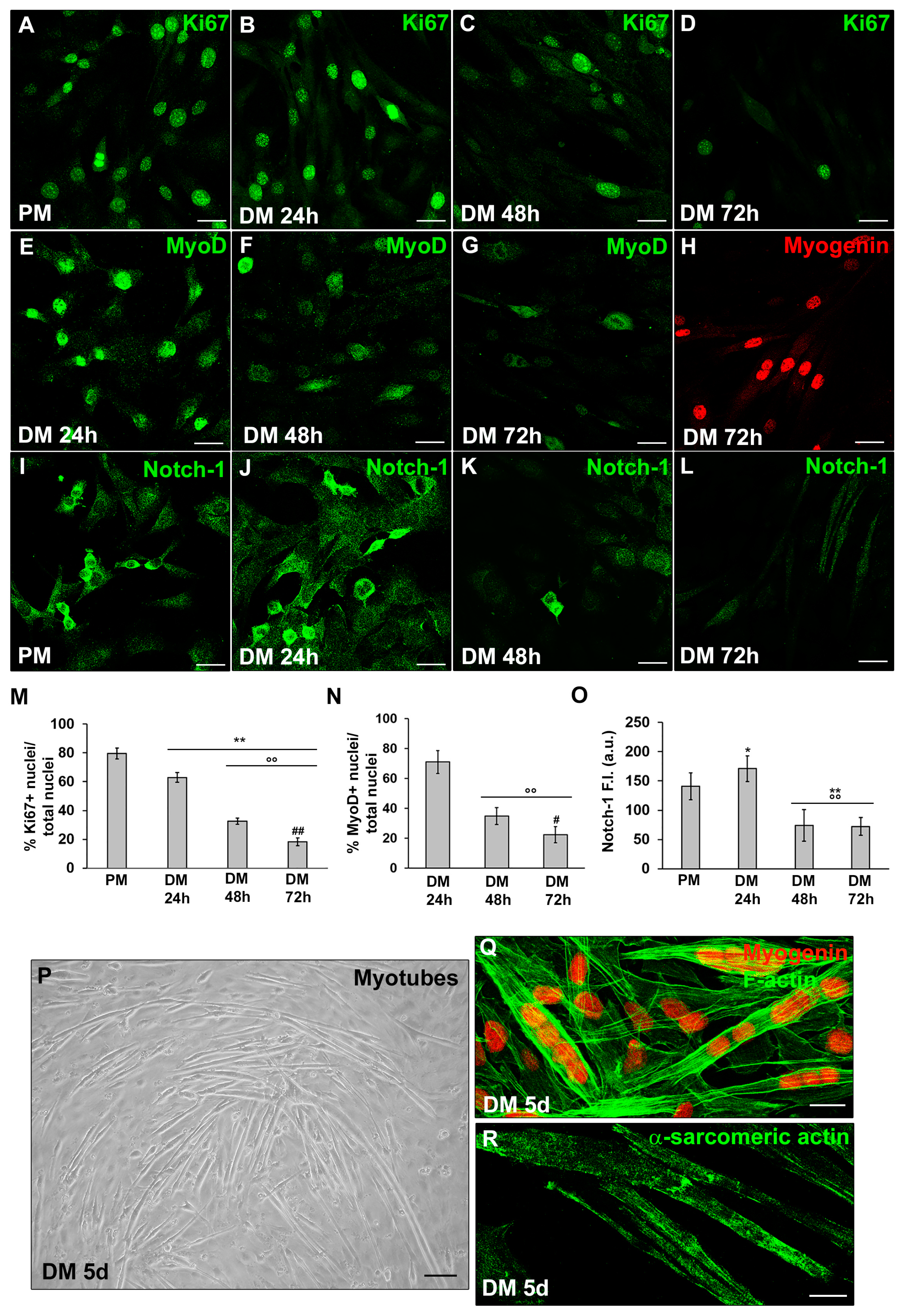
Figure 2.
Evaluation of the SDH-B, LDH-A and PGC-1α during C2C12 myoblast differentiation. The cells were cultured in proliferation medium (PM) for 24h or in myogenic differentiation medium (DM) for different times (24, 48, 72h and 5d). (A,B) Western Blotting analysis of SDH-B, LDH-A: representative blot and bar charts showing the densitometric analysis of the bands normalized to α-tubulin. (C–G) Representative confocal fluorescence images of the cells immunostained with antibodies against PGC-1α (green). Scale bar: 25 µm. (H) Bar charts showing the densitometric analysis of the PGC-1α fluorescent signal intensity (F.I.) performed on digitized images in 10 regions of interest (ROI) of 100 μm2 for each confocal stack (10). a.u.: arbitrary units. Data shown are mean ± SD and represent the results of at least three independent experiments performed in triplicate. Significance of difference: * p<0.05, ** p<0.01 vs PM; °° p<0.01 vs DM 24h; # p<0.05, ## p<0.01 vs DM 48h (One-way ANOVA with post-hoc Tukey HSD).
Figure 2.
Evaluation of the SDH-B, LDH-A and PGC-1α during C2C12 myoblast differentiation. The cells were cultured in proliferation medium (PM) for 24h or in myogenic differentiation medium (DM) for different times (24, 48, 72h and 5d). (A,B) Western Blotting analysis of SDH-B, LDH-A: representative blot and bar charts showing the densitometric analysis of the bands normalized to α-tubulin. (C–G) Representative confocal fluorescence images of the cells immunostained with antibodies against PGC-1α (green). Scale bar: 25 µm. (H) Bar charts showing the densitometric analysis of the PGC-1α fluorescent signal intensity (F.I.) performed on digitized images in 10 regions of interest (ROI) of 100 μm2 for each confocal stack (10). a.u.: arbitrary units. Data shown are mean ± SD and represent the results of at least three independent experiments performed in triplicate. Significance of difference: * p<0.05, ** p<0.01 vs PM; °° p<0.01 vs DM 24h; # p<0.05, ## p<0.01 vs DM 48h (One-way ANOVA with post-hoc Tukey HSD).
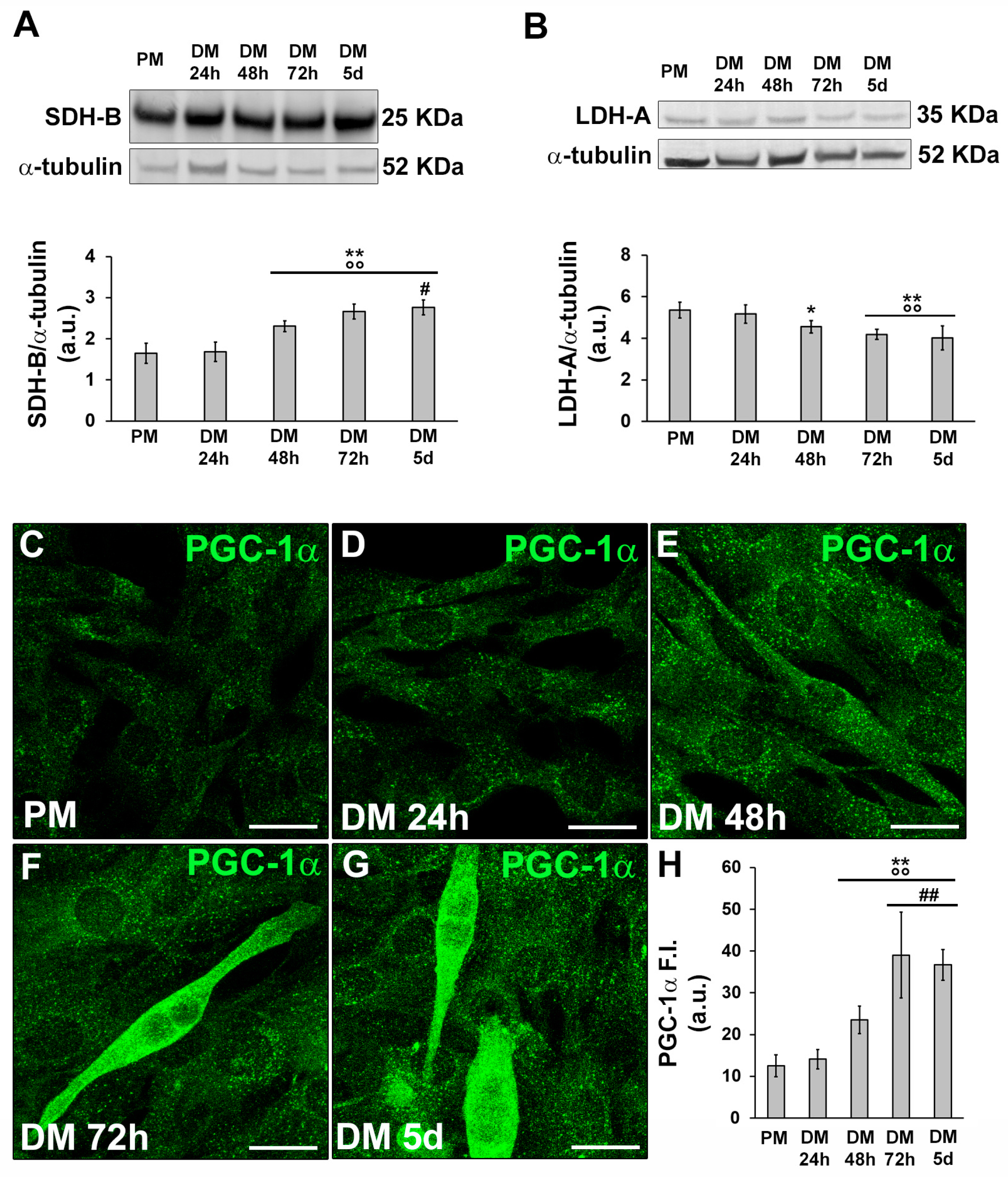
Figure 3.
Evaluation of HIF-1α expression and cellular localization during C2C12 myoblast differentiation. The cells were cultured in proliferation medium (PM) for 24h or in myogenic differentiation medium (DM) for different times (24, 48, 72h and 5d). (A) RT-PCR analysis. Representative agarose gel and bar charts showing the densitometric analysis of the bands normalized to β-actin. a.u.: arbitrary units. (B) Western Blotting analysis. Representative blot and bar charts showing the densitometric analysis of the bands normalized to α-tubulin. Data shown are mean ± SD and represent the results of at least three independent experiments performed in triplicate. (C) Representative confocal fluorescence images of the cells immunostained with antibodies against HIF-1α (green). Note the nuclear localization of the factor in the early differentiating cells. Scale bar: 25 µm. Significance of difference: ** p<0.01 vs PM; °° p<0.01 vs DM 24h; ## p<0.01 vs DM 48h (One-way ANOVA with post-hoc Tukey HSD).
Figure 3.
Evaluation of HIF-1α expression and cellular localization during C2C12 myoblast differentiation. The cells were cultured in proliferation medium (PM) for 24h or in myogenic differentiation medium (DM) for different times (24, 48, 72h and 5d). (A) RT-PCR analysis. Representative agarose gel and bar charts showing the densitometric analysis of the bands normalized to β-actin. a.u.: arbitrary units. (B) Western Blotting analysis. Representative blot and bar charts showing the densitometric analysis of the bands normalized to α-tubulin. Data shown are mean ± SD and represent the results of at least three independent experiments performed in triplicate. (C) Representative confocal fluorescence images of the cells immunostained with antibodies against HIF-1α (green). Note the nuclear localization of the factor in the early differentiating cells. Scale bar: 25 µm. Significance of difference: ** p<0.01 vs PM; °° p<0.01 vs DM 24h; ## p<0.01 vs DM 48h (One-way ANOVA with post-hoc Tukey HSD).
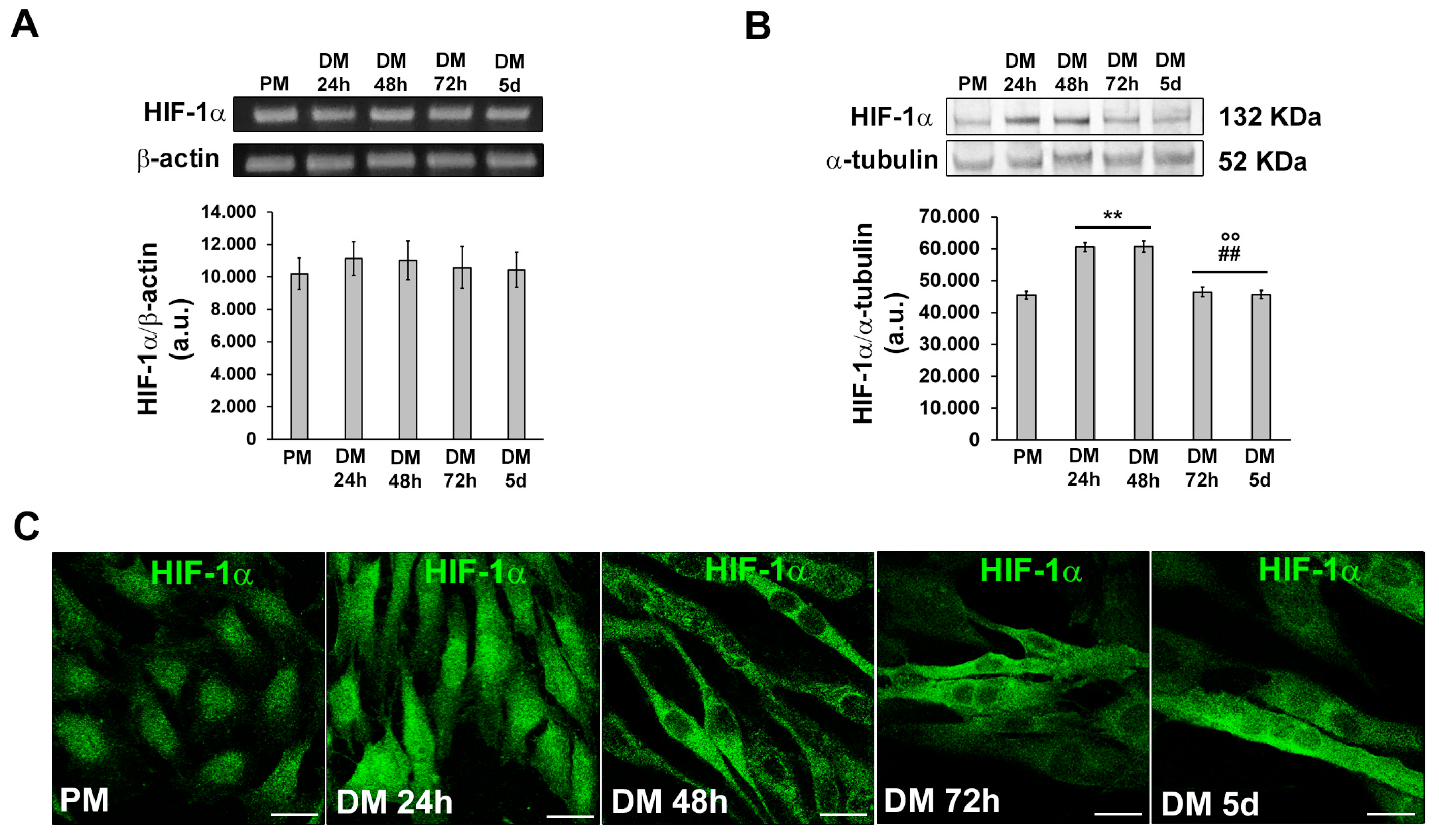
Figure 4.
Evaluation of MMP-9 expression and cellular localization during C2C12 myoblast differentiation. The cells were cultured in proliferation medium (PM) for 24h or in myogenic differentiation medium (DM) for different times (24, 48, 72h and 5d). (A) RT-PCR analysis. Representative agarose gel and bar charts showing the densitometric analysis of the bands normalized to β-actin. a.u.: arbitrary units. (B) Western Blotting analysis. Representative blot and bar charts showing the densitometric analysis of the bands normalized to α-tubulin. (C-G) Representative confocal fluorescence images of the cells immunostained with antibodies against MMP-9 (green). Scale bar: 25 µm. (H) Bar charts showing the densitometric analysis of the MMP-9 fluorescent signal intensity (F.I.) performed on digitized images in 10 regions of interest (ROI) of 100 μm2 for each confocal stack (10). a.u.: arbitrary units. Data shown are mean ± SD and represent the results of at least three independent experiments performed in triplicate. Significance of difference: ** p<0.01 vs PM; °° p<0.01 vs DM 24h; # p<0.05, ## p<0.01 vs DM 48h (One-way ANOVA with post-hoc Tukey HSD).
Figure 4.
Evaluation of MMP-9 expression and cellular localization during C2C12 myoblast differentiation. The cells were cultured in proliferation medium (PM) for 24h or in myogenic differentiation medium (DM) for different times (24, 48, 72h and 5d). (A) RT-PCR analysis. Representative agarose gel and bar charts showing the densitometric analysis of the bands normalized to β-actin. a.u.: arbitrary units. (B) Western Blotting analysis. Representative blot and bar charts showing the densitometric analysis of the bands normalized to α-tubulin. (C-G) Representative confocal fluorescence images of the cells immunostained with antibodies against MMP-9 (green). Scale bar: 25 µm. (H) Bar charts showing the densitometric analysis of the MMP-9 fluorescent signal intensity (F.I.) performed on digitized images in 10 regions of interest (ROI) of 100 μm2 for each confocal stack (10). a.u.: arbitrary units. Data shown are mean ± SD and represent the results of at least three independent experiments performed in triplicate. Significance of difference: ** p<0.01 vs PM; °° p<0.01 vs DM 24h; # p<0.05, ## p<0.01 vs DM 48h (One-way ANOVA with post-hoc Tukey HSD).

Figure 5.
HIF-1α and MMP-9 expression in satellite cells (SCs). A murine SC-enriched culture was obtained from single skeletal EDL muscle fiber as reported in Material and methods. The cells were cultured for 24 h and 48 h in their specific SC proliferation medium. Representative immunofluorescence confocal images of fixed SCs in the indicated experimental conditions, immunostained with (A,B) antibodies against Pax7 (red) and HIF-1α (green), (C,D) antibodies against Pax7 (red) and MMP-9 (green).
Figure 5.
HIF-1α and MMP-9 expression in satellite cells (SCs). A murine SC-enriched culture was obtained from single skeletal EDL muscle fiber as reported in Material and methods. The cells were cultured for 24 h and 48 h in their specific SC proliferation medium. Representative immunofluorescence confocal images of fixed SCs in the indicated experimental conditions, immunostained with (A,B) antibodies against Pax7 (red) and HIF-1α (green), (C,D) antibodies against Pax7 (red) and MMP-9 (green).
Figure 6.
Evaluation of HIF-1α silencing on C2C12 myoblast MyoD, Notch-ICD and MMP-9 expression and morphology. The cells were transfected in the proliferation medium (PM) with either scrambled siRNA (SCR-siRNA) or HIF-1α-siRNA. After transfection -PM (T0)- the cells were induced to differentiate by shifting in the differentiation medium (DM) for 24 h. WT: wild type not transfected cells. (A,B) Western Blotting analysis. Representative blot and bar charts showing the densitometric analysis of the bands normalized to α-tubulin. (C) Representative confocal fluorescence images of the cells immunostained with antibodies against HIF-1α (green). Scale bar: 25 µm. Bar charts showing the densitometric analysis of the HIF-1α fluorescent signal intensity (F.I.) performed on digitized images in 10 regions of interest (ROI) of 100 μm2 for each confocal stack (10). a.u.: arbitrary units. Data shown are mean ± SD and represent the results of at least three independent experiments performed in triplicate. Significance of difference: ** p<0.01 vs WT PM (T0); ° p<0.05, °° p<0.01 vs SCR-siRNA PM (T0); ## p<0.01 vs WT DM 24h; §§ p<0.01 vs SCR-siRNA DM 24h (One-way ANOVA with post-hoc Tukey HSD). (D) Representative differential interference contrast (DIC) images of fixed C2C12 cells in the indicated experimental conditions.
Figure 6.
Evaluation of HIF-1α silencing on C2C12 myoblast MyoD, Notch-ICD and MMP-9 expression and morphology. The cells were transfected in the proliferation medium (PM) with either scrambled siRNA (SCR-siRNA) or HIF-1α-siRNA. After transfection -PM (T0)- the cells were induced to differentiate by shifting in the differentiation medium (DM) for 24 h. WT: wild type not transfected cells. (A,B) Western Blotting analysis. Representative blot and bar charts showing the densitometric analysis of the bands normalized to α-tubulin. (C) Representative confocal fluorescence images of the cells immunostained with antibodies against HIF-1α (green). Scale bar: 25 µm. Bar charts showing the densitometric analysis of the HIF-1α fluorescent signal intensity (F.I.) performed on digitized images in 10 regions of interest (ROI) of 100 μm2 for each confocal stack (10). a.u.: arbitrary units. Data shown are mean ± SD and represent the results of at least three independent experiments performed in triplicate. Significance of difference: ** p<0.01 vs WT PM (T0); ° p<0.05, °° p<0.01 vs SCR-siRNA PM (T0); ## p<0.01 vs WT DM 24h; §§ p<0.01 vs SCR-siRNA DM 24h (One-way ANOVA with post-hoc Tukey HSD). (D) Representative differential interference contrast (DIC) images of fixed C2C12 cells in the indicated experimental conditions.
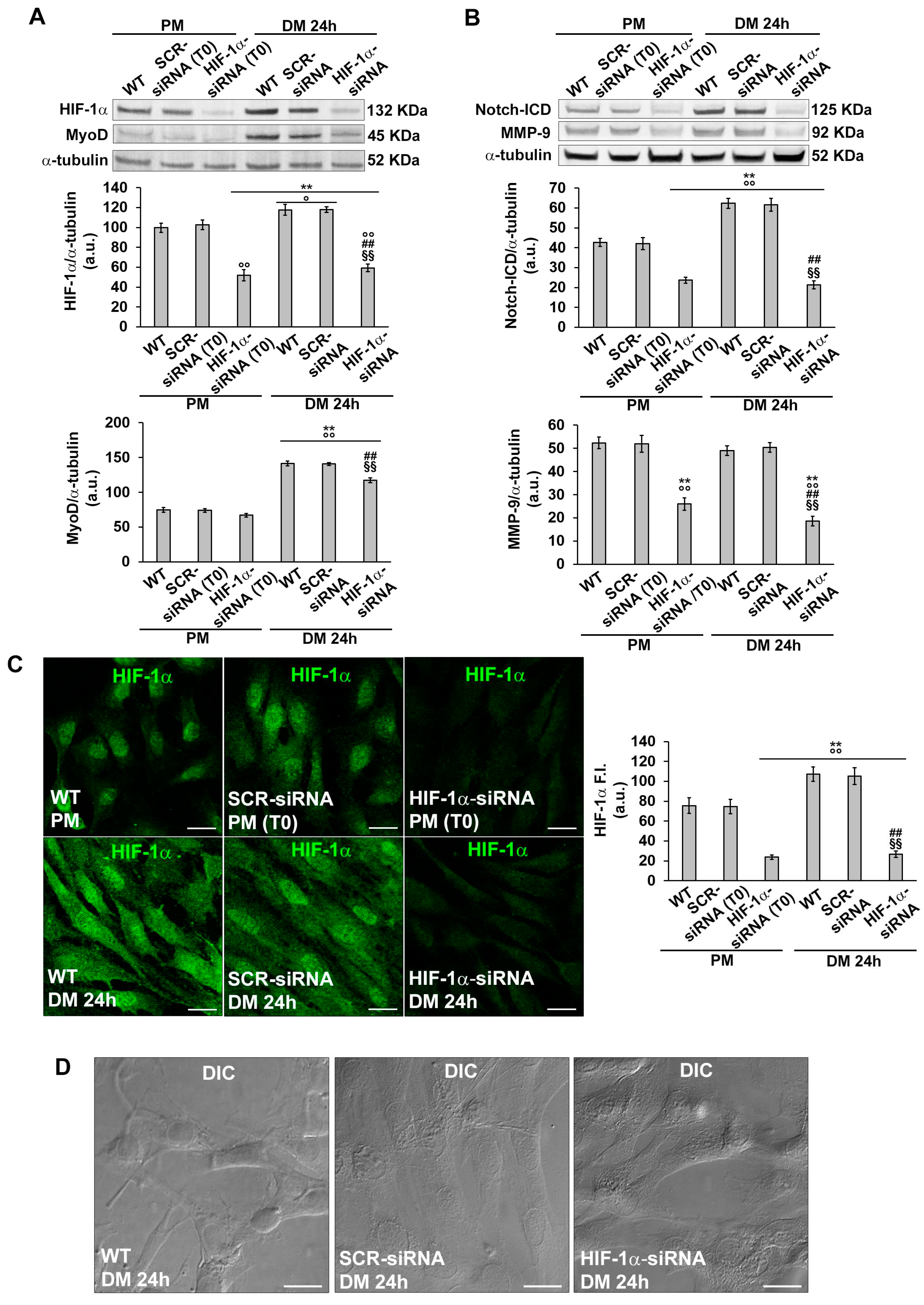
Figure 7.
Evaluation of HIF-1α silencing on C2C12 myoblast Ki67, MyoD, Notch-1 and MMP-9 expression. The cells were transfected with either scrambled siRNA (SCR-siRNA) or HIF-1α-siRNA in PM (T0). After transfection the cells were induced to differentiate by shifting in the differentiation medium (DM) for 24 h. WT: wild type not transfected cells. (A-C,E-GI-K,M-O) Representative confocal fluorescence images of the cells immunostained with antibodies against the indicated markers (green). Scale bar: 25 µm. (D,H) Quantitative analyses of Ki67 and MyoD positive nuclei expressed as the percentage of the total nuclei. (L,P) Bar charts showing densitometric analysis of the Notch-1 and MMP-9 fluorescent signal intensity (F.I.) performed on digitized images in 10 regions of interest (ROI) of 100 μm2 for each confocal stack (10). a.u.: arbitrary units. Data shown are mean ± SD and represent the results of at least three independent experiments performed in triplicate. Significance of difference: ** p<0.01 vs WT DM 24h; °° p<0.01 vs SCR-siRNA DM 24h (One-way ANOVA with post-hoc Tukey HSD).
Figure 7.
Evaluation of HIF-1α silencing on C2C12 myoblast Ki67, MyoD, Notch-1 and MMP-9 expression. The cells were transfected with either scrambled siRNA (SCR-siRNA) or HIF-1α-siRNA in PM (T0). After transfection the cells were induced to differentiate by shifting in the differentiation medium (DM) for 24 h. WT: wild type not transfected cells. (A-C,E-GI-K,M-O) Representative confocal fluorescence images of the cells immunostained with antibodies against the indicated markers (green). Scale bar: 25 µm. (D,H) Quantitative analyses of Ki67 and MyoD positive nuclei expressed as the percentage of the total nuclei. (L,P) Bar charts showing densitometric analysis of the Notch-1 and MMP-9 fluorescent signal intensity (F.I.) performed on digitized images in 10 regions of interest (ROI) of 100 μm2 for each confocal stack (10). a.u.: arbitrary units. Data shown are mean ± SD and represent the results of at least three independent experiments performed in triplicate. Significance of difference: ** p<0.01 vs WT DM 24h; °° p<0.01 vs SCR-siRNA DM 24h (One-way ANOVA with post-hoc Tukey HSD).

Figure 8.
Evaluation of MMP-9 silencing or inhibition on C2C12 myoblast MyoD, Notch-1 and Ki67 expression and morphology. The cells were transfected in the proliferation medium (PM) with either scrambled siRNA (SCR-siRNA) or MMP-9-siRNA. After transfection - PM (T0) - the cells were induced to differentiate by shifting in the differentiation medium (DM) for 24 h. In some experiments the cells were cultured in DM in the presence of the MMP-9 inhibitor SB-3CT (10 µM). WT: wild type not-transfected or untreated cells. (A) Western Blotting analysis. Representative blot and bar charts showing the densitometric analysis of the bands normalized to α-tubulin. (B-E) Representative differential interference contrast (DIC) images of fixed C2C12 cells in the indicated experimental conditions. Scale bar: 12.5 µm. (F-H,J-U) Representative confocal fluorescence images of the cells immunostained with antibodies against the indicated markers (green). Scale bar: 25 µm. (I,X) Bar charts showing the densitometric analysis of the MMP-9 and Notch-1 fluorescent signal intensity (F.I.) performed on digitized images in 10 regions of interest (ROI) of 100 μm2 for each confocal stack (10). a.u.: arbitrary units. (V,W) Quantitative analyses of Ki67 and MyoD positive nuclei expressed as the percentage of the total nuclei, respectively. Data shown are mean ± SD and represent the results of at least three independent experiments performed in triplicate. Significance of difference: in (A): * p<0.05, ** p<0.01 vs WT PM; ° p<0.05, °° p<0.01 vs SCR-siRNA PM (T0); ## p<0.01 vs WT DM 24h; §§ p<0.01 vs SCR-siRNA DM 24h. In (I,V-X): ** p<0.01 vs WT; °° p<0.01 vs SCR-siRNA (One-way ANOVA with post-hoc Tukey HSD).
Figure 8.
Evaluation of MMP-9 silencing or inhibition on C2C12 myoblast MyoD, Notch-1 and Ki67 expression and morphology. The cells were transfected in the proliferation medium (PM) with either scrambled siRNA (SCR-siRNA) or MMP-9-siRNA. After transfection - PM (T0) - the cells were induced to differentiate by shifting in the differentiation medium (DM) for 24 h. In some experiments the cells were cultured in DM in the presence of the MMP-9 inhibitor SB-3CT (10 µM). WT: wild type not-transfected or untreated cells. (A) Western Blotting analysis. Representative blot and bar charts showing the densitometric analysis of the bands normalized to α-tubulin. (B-E) Representative differential interference contrast (DIC) images of fixed C2C12 cells in the indicated experimental conditions. Scale bar: 12.5 µm. (F-H,J-U) Representative confocal fluorescence images of the cells immunostained with antibodies against the indicated markers (green). Scale bar: 25 µm. (I,X) Bar charts showing the densitometric analysis of the MMP-9 and Notch-1 fluorescent signal intensity (F.I.) performed on digitized images in 10 regions of interest (ROI) of 100 μm2 for each confocal stack (10). a.u.: arbitrary units. (V,W) Quantitative analyses of Ki67 and MyoD positive nuclei expressed as the percentage of the total nuclei, respectively. Data shown are mean ± SD and represent the results of at least three independent experiments performed in triplicate. Significance of difference: in (A): * p<0.05, ** p<0.01 vs WT PM; ° p<0.05, °° p<0.01 vs SCR-siRNA PM (T0); ## p<0.01 vs WT DM 24h; §§ p<0.01 vs SCR-siRNA DM 24h. In (I,V-X): ** p<0.01 vs WT; °° p<0.01 vs SCR-siRNA (One-way ANOVA with post-hoc Tukey HSD).

Figure 9.
Electrophysiological analysis. The cells were transfected in the proliferation medium (PM) with either scrambled siRNA (SCR-siRNA) or HIF-1α-siRNA. After transfection -PM (T0)- the cells were induced to differentiate by shifting in the differentiation medium (DM) for 24 h. WT: wild type, not-transfected or untreated cells. In other experiments the cells were cultured in PM or DM in the presence of the MMP-9 inhibitor SB-3CT (10 µM) for 24 h. (A) Pulse protocol of stimulation in voltage clamp (top) and typical tracings of passive membrane currents (bottom) obtained in response to its application. (B) Cell capacitance (Cm, in pF) as an index of cell surface. (C) Specific membrane conductance, Gm/Cm (in nS/pF). Data are mean ± SD. * p<0.05 vs WT PM, # p<0.05 vs WT DM 24h (One way ANOVA followed by Bonferroni's post hoc test).
Figure 9.
Electrophysiological analysis. The cells were transfected in the proliferation medium (PM) with either scrambled siRNA (SCR-siRNA) or HIF-1α-siRNA. After transfection -PM (T0)- the cells were induced to differentiate by shifting in the differentiation medium (DM) for 24 h. WT: wild type, not-transfected or untreated cells. In other experiments the cells were cultured in PM or DM in the presence of the MMP-9 inhibitor SB-3CT (10 µM) for 24 h. (A) Pulse protocol of stimulation in voltage clamp (top) and typical tracings of passive membrane currents (bottom) obtained in response to its application. (B) Cell capacitance (Cm, in pF) as an index of cell surface. (C) Specific membrane conductance, Gm/Cm (in nS/pF). Data are mean ± SD. * p<0.05 vs WT PM, # p<0.05 vs WT DM 24h (One way ANOVA followed by Bonferroni's post hoc test).
Figure 10.
Ca2+ current evaluation in C2C12 cells. Representative time course of Ca2+ currents normalized for cell capacitance (in pA/pF) from (A) a wild type (WT) C2C12 cell cultured in proliferation medium PM or (E) differentiation medium DM for 24 h; (B) from a scrambled siRNA (SCR-siRNA) transfected cell cultured in PM (T0) or (F) in DM for 24 h; (C) from a HIF-1α-siRNA transfected cell cultured in PM (T0) or (G) in DM for 24 h; (D) from a cell treated with the MMP-9 inhibitor SB-3CT (10 µM) for 24 h in PM or (H) in DM . (I) Pulse protocol of stimulation used to evoke ion currents. (J) Overall I-V relation related to the four conditions for all the experiments done in PM (WT PM, n=7, open circles ; SCR-siRNA PM (T0) n=4, open squares; HIF-1α-siRNA PM (T0) n=4, open triangles; SB-3CT PM 24h n=6, open diamonds) and (K) in DM (WT DM 24h n=6, gray circles; SCR-siRNA DM 24h n=2, gray squares; HIF-1α-siRNA DM 24h n=3, gray triangles; SB-3CT DM 24h n=7, gray diamonds). Data are mean ± SD. (p>0.05 two-way ANOVA).
Figure 10.
Ca2+ current evaluation in C2C12 cells. Representative time course of Ca2+ currents normalized for cell capacitance (in pA/pF) from (A) a wild type (WT) C2C12 cell cultured in proliferation medium PM or (E) differentiation medium DM for 24 h; (B) from a scrambled siRNA (SCR-siRNA) transfected cell cultured in PM (T0) or (F) in DM for 24 h; (C) from a HIF-1α-siRNA transfected cell cultured in PM (T0) or (G) in DM for 24 h; (D) from a cell treated with the MMP-9 inhibitor SB-3CT (10 µM) for 24 h in PM or (H) in DM . (I) Pulse protocol of stimulation used to evoke ion currents. (J) Overall I-V relation related to the four conditions for all the experiments done in PM (WT PM, n=7, open circles ; SCR-siRNA PM (T0) n=4, open squares; HIF-1α-siRNA PM (T0) n=4, open triangles; SB-3CT PM 24h n=6, open diamonds) and (K) in DM (WT DM 24h n=6, gray circles; SCR-siRNA DM 24h n=2, gray squares; HIF-1α-siRNA DM 24h n=3, gray triangles; SB-3CT DM 24h n=7, gray diamonds). Data are mean ± SD. (p>0.05 two-way ANOVA).
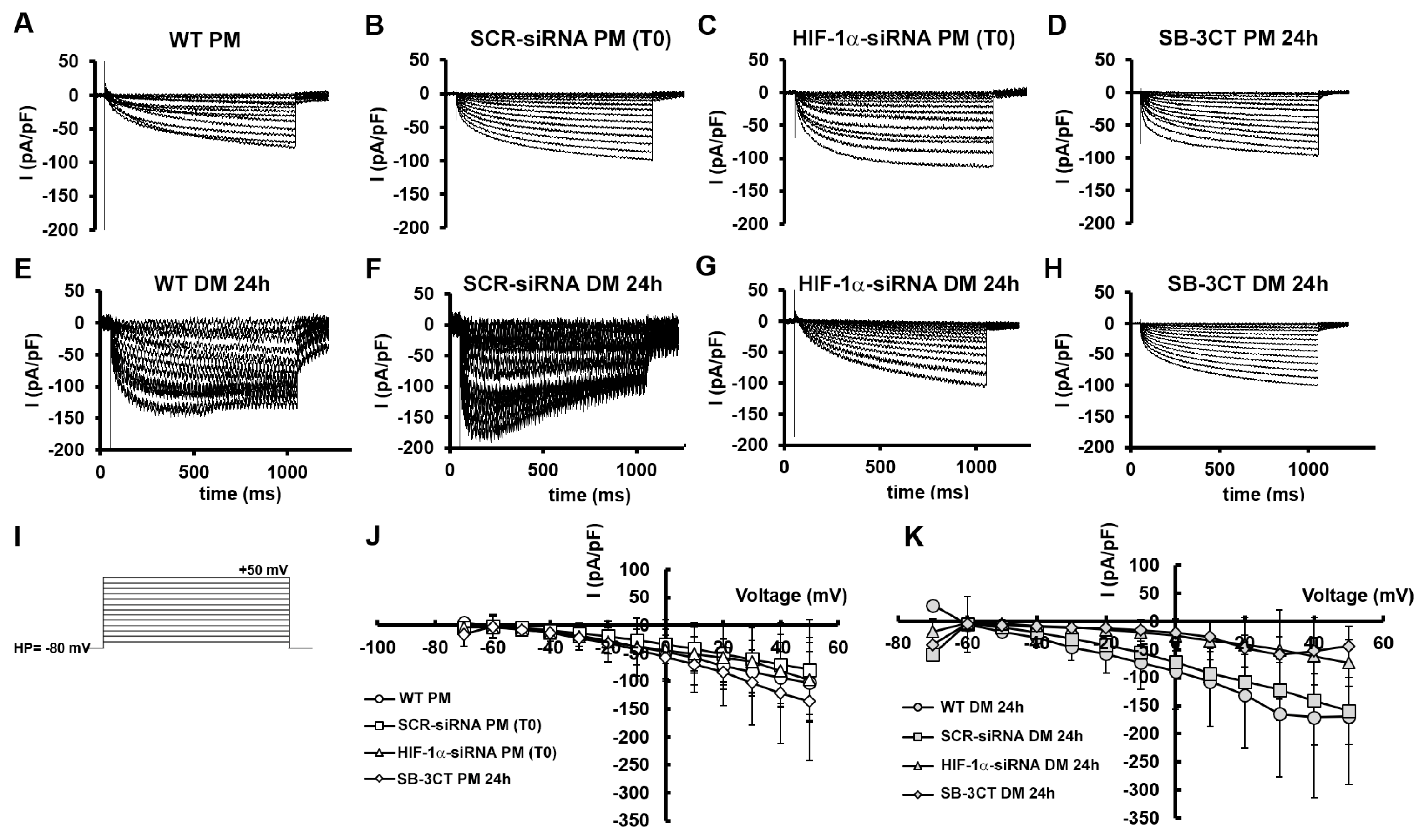
Table 1.
Specifications of primary antibodies used for immunofluorescence (IF) and Western Blotting (WB) analyses.
Table 1.
Specifications of primary antibodies used for immunofluorescence (IF) and Western Blotting (WB) analyses.
Primary Antibody name |
Company |
Code |
Dilution |
anti-Ki67 rabbit polyclonal |
Abcam, Cambridge, UK |
ab15580 |
1:100 (IF) |
anti-Notch-1 rabbit monoclonal |
Abcam |
ab52627 |
1:100 (IF) 1:1000 (WB) |
anti-MyoD (M-318) rabbit polyclonal |
Santa Cruz Biotechnology |
sc-760 |
1:50 (IF) 1:500 (WB) |
anti-myogenin (F5D) mouse monoclonal |
Santa Cruz Biotechnology |
sc-12732 |
1:50 (IF) |
anti-α-sarcomeric actin mouse monoclonal |
DakoCytomation, Carpinteria, CA, USA
|
M 0874 |
1:100 (IF) |
anti- peroxisome proliferator-activated receptor-gamma coactivator (PGC)-1α mouse monoclonal |
Santa Cruz Biotechnology |
sc-518025 |
1:100 (IF) |
anti-HIF-1α rabbit polyclonal |
Santa Cruz Biotechnology |
sc-10790 |
1:100 (IF) 1:1000 (WB) |
anti-MMP-9 rabbit polyclonal |
Bioss Antibodies, Woburn, MA, USA |
bs-0397R |
1:100 (IF) 1:1000 (WB) |
anti-Pax7 mouse monoclonal |
Santa Cruz Biotechnology |
sc-81648 |
1:100 (IF)
|
anti-succinate dehydrogenase complex iron sulfur subunit-B (SDH-B) rabbit polyclonal |
Sigma-Aldrich |
SAB2102103 |
1:1000 (WB) |
anti-lactate dehydrogenase (LDH)-A (E-9) mouse monoclonal |
Santa Cruz Biotechnology |
sc-137243 |
1:1000 (WB) |
anti-α-tubulin rabbit polyclonal |
GeneTex, Prodotti Gianni, Milano, Italy |
GTX112141 |
1:1000 (WB) |
Table 2.
C2C12 cells passive membrane properties. The cells were treated and cultured as indicated in
Figure 9. At least three independent C2C12 cells culture preparation were analyzed. n: number of the cells. Data are mean ± SD. * p<0.05 vs WT PM; # p<0.05 vs WT DM 24h (One way ANOVA followed by Bonferroni's
post hoc test).
Table 2.
C2C12 cells passive membrane properties. The cells were treated and cultured as indicated in
Figure 9. At least three independent C2C12 cells culture preparation were analyzed. n: number of the cells. Data are mean ± SD. * p<0.05 vs WT PM; # p<0.05 vs WT DM 24h (One way ANOVA followed by Bonferroni's
post hoc test).
Condition |
Cm (pF) |
Gm/Cm (nS/pF) |
WT PM |
23.56±4.20 n=5 |
1.29×10-2±0.77×10-2 n=10 |
SCR-siRNA PM (T0)
|
15.51±4.43 n=3 |
2.18×10-2±1.79×10-2 n=4 |
HIF-1α-siRNA PM (T0)
|
19.65±6.68 n=4 |
2.82×10-2±1.97×10-2 n=4 |
SB-3CT PM 24h |
25.08±17.06 n=6 |
3.78×10-2±3.10×10-2 n=5 |
WT DM 24h |
41.87±19.62* n=10 |
3.04×10-2±2.21×10-2* n=10 |
SCR-siRNA DM 24h |
40.58±25.22* n=7 |
4.11×10-2±2,09×10-2* n=6 |
HIF-1α-siRNA DM 24h |
20.08±7.02# n=5 |
1.27×10-2±1.03×10-2# n=11 |
SB-3CT DM 24h |
19.68±10.81# n=5 |
0.93×10-2±0.82×10-2# n=4 |